Magnetic Fields
No discussion of electromagnetism would be complete without, well, magnetism. Magnetism is the irreplaceable partner to electricity—the Starsky to its Hutch, the Siegfried to its Roy, the Ferb to its Phineas. And really, magnetism and electricity are two ways to describe the same phenomena: that of electromagnetism. One's not complete without the other.
Any moving charge creates a magnetic field. This means large scale currents or electron beams are all magnetic to some degree, but even moving charge on the smallest possible scale—electron orbitals—can create strong magnetic fields. Any ferromagnetic material, such as iron, can form a permanent magnet like the ones on your fridge if all of its electrons' orbits are aligned just the right way, but has no current in it like what we would create by attaching a wire to a battery.
Unlike electric fields, magnetic fields have no point sources—there's no such thing as a magnetic charge. Magnetic fields do have a polarity, called north and south, and any magnet will have a north end and a south end (called poles). Split the magnet in half, and each half will still have a north end and a south end. Like positive and negative charge, the north pole of a magnet repels other norths and is attracted to souths. The south pole repels other souths and is attracted to norths. (Magnets would have ended the Civil War a lot faster than America did.)
We draw magnetic field lines as traveling from north to south. In a common bar magnet, that would look like this:
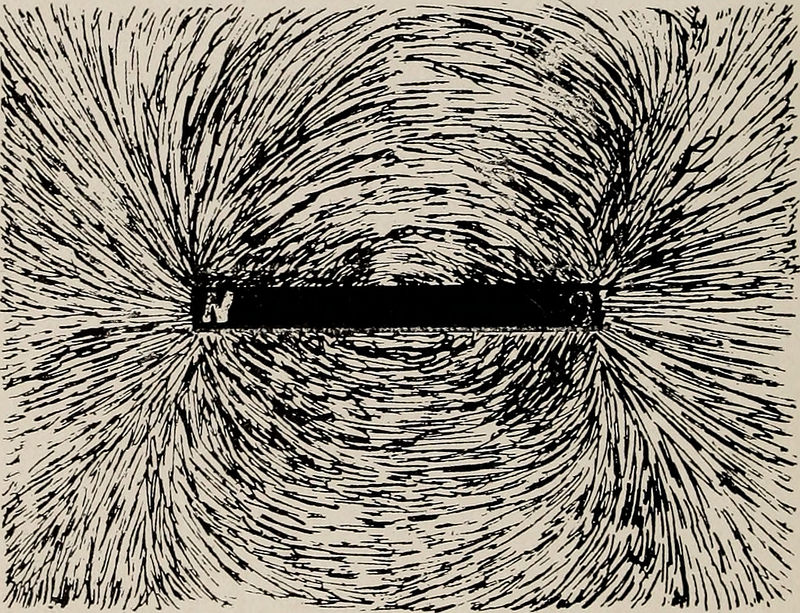
Trippy
(Source)
In fact, we can prove this to ourselves by taking a bunch of iron filings, scattering them on a table, and placing a bar magnet on the table—the iron aligns itself to the magnet's previously invisible magnetic field.
While electric fields radiate outward from charges, magnetic fields behave quite differently. The magnetic field of a current-carrying wire will circle around the wire, though like electric field it still gets weaker as you get further away from the wire.
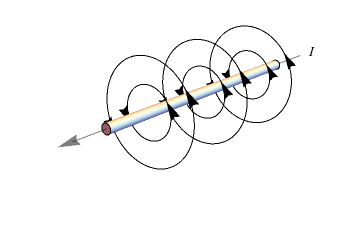
Because physicists like to draw in two dimensions, we will often draw current-carrying wires coming into the screen (⊗) or out of the screen (⊙) so that their fields circles around on the plane of the screen. Much easier to draw.
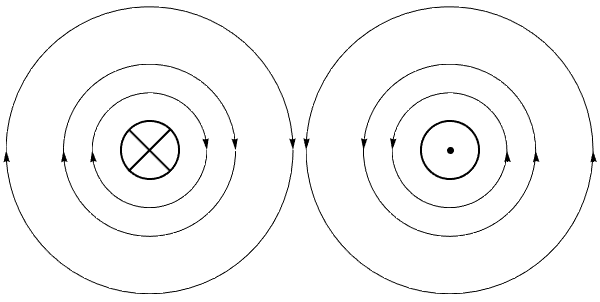
We can determine which direction the magnetic field circles using right hand rule #1: point your thumb in the direction of the current and curl your fingers around—your fingers point in the same direction as the magnetic field created by the current.
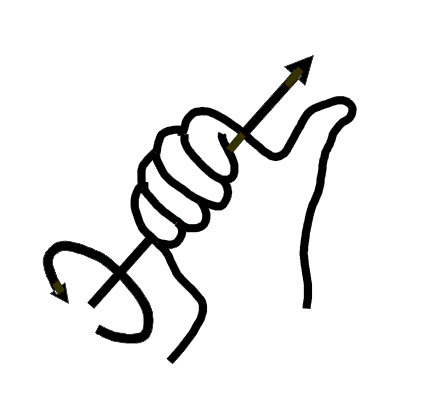
Thumbs up, dude.
(Source)
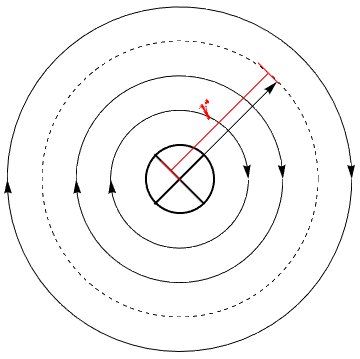
The magnetic field created by the enclosed current is:
Bl = μ 0I
Here, l is the length of the section of our loop that is parallel to the magnetic field. The symbol μ0 represents the magnetic permeability of free space. It is exactly analogous to electric permittivity—μ0 represents the ease a magnetic field has moving through vacuum (or, as with ε0, air). Permeability is measured in N/A2, where:
μ0 = 4π × 10-7 N/A2
This means that magnetic fields are measured in
, which is defined as a tesla (T). One tesla is quite large—Earth's magnetic field is around 30 μT at the equator, refrigerator magnets create a field of just a few millitesla, and even strong loudspeaker magnets only go up to one or two tesla.
For the case of an Ampèrian loop drawn around a current carrying wire, both the loop and the field are circular, so the loop is parallel to the field at every single point. In this case, l is equal to the entire circumference of the loop and we can write:
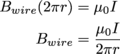
Electromagnetic radiation comes in many different forms—in fact, many different forms you've heard of: radio waves, microwaves, infrared light, visible light, ultraviolet light, X-rays, and gamma rays. These are all forms of electromagnetic energy. And in vacuum, each of them travels at the speed of light (c). Well, guess what the speed of light is equal to:

In future chapters, we'll learn more about what a wave is in physics and how waves move. For now, let's just say waves are what happen when a charged particle listens to too many Beach Boys albums. The important things to note are:
• Electromagnetic waves are a form of electromagnetic energy that is radiated from charged particles.
• The amount of electromagnetic energy in a wave is given by its frequency, a measure of how quickly the wave moves up and down. Frequency is measured in inverse seconds, or hertz (Hz).
We group waves by frequency in a chart called the electromagnetic spectrum.
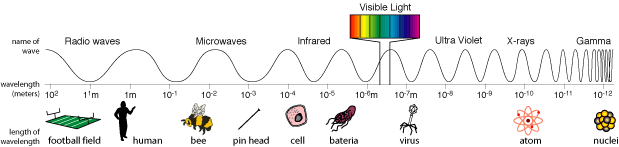
Everything you'd like to see…and then some.
(Source)
The higher the frequency, the more energy is in the wave. Radio waves are harmless, but microwaves can cook food, X-rays can see through skin and muscle, and gamma rays—well, Hulk smash. Sitting in the middle of the EM spectrum is visible light, how humans perceive the world around us. Low frequency light looks red or orange, while higher frequency light looks blue or violet.
Any moving charge creates a magnetic field. This means large scale currents or electron beams are all magnetic to some degree, but even moving charge on the smallest possible scale—electron orbitals—can create strong magnetic fields. Any ferromagnetic material, such as iron, can form a permanent magnet like the ones on your fridge if all of its electrons' orbits are aligned just the right way, but has no current in it like what we would create by attaching a wire to a battery.
Unlike electric fields, magnetic fields have no point sources—there's no such thing as a magnetic charge. Magnetic fields do have a polarity, called north and south, and any magnet will have a north end and a south end (called poles). Split the magnet in half, and each half will still have a north end and a south end. Like positive and negative charge, the north pole of a magnet repels other norths and is attracted to souths. The south pole repels other souths and is attracted to norths. (Magnets would have ended the Civil War a lot faster than America did.)
We draw magnetic field lines as traveling from north to south. In a common bar magnet, that would look like this:
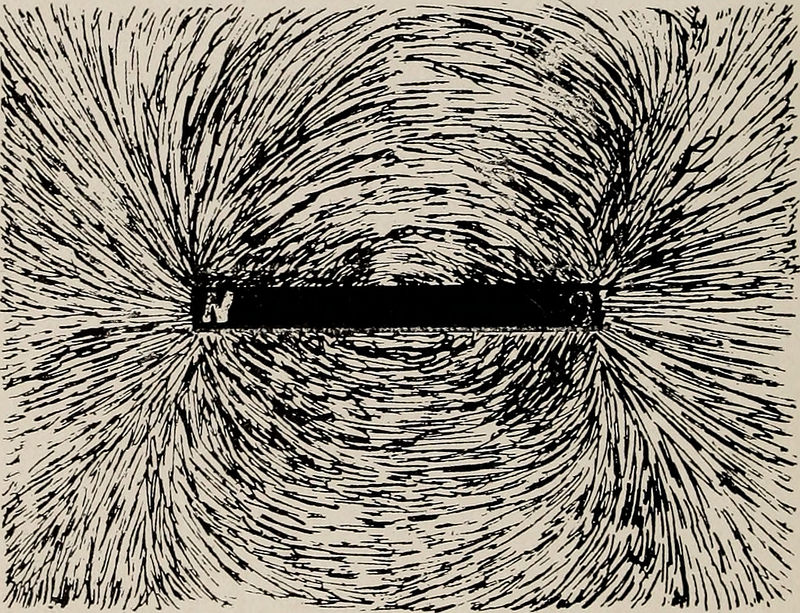
Trippy
(Source)
In fact, we can prove this to ourselves by taking a bunch of iron filings, scattering them on a table, and placing a bar magnet on the table—the iron aligns itself to the magnet's previously invisible magnetic field.
While electric fields radiate outward from charges, magnetic fields behave quite differently. The magnetic field of a current-carrying wire will circle around the wire, though like electric field it still gets weaker as you get further away from the wire.
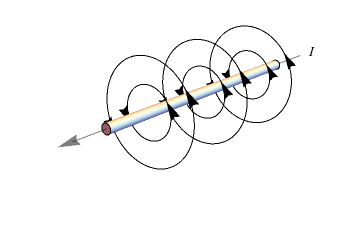
Because physicists like to draw in two dimensions, we will often draw current-carrying wires coming into the screen (⊗) or out of the screen (⊙) so that their fields circles around on the plane of the screen. Much easier to draw.
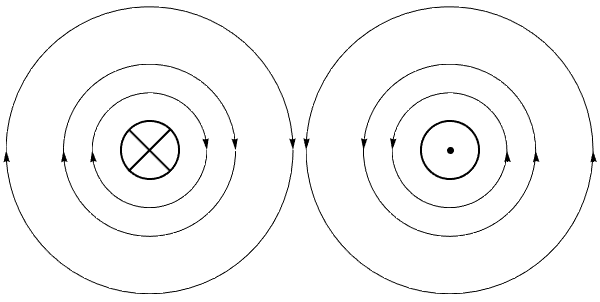
We can determine which direction the magnetic field circles using right hand rule #1: point your thumb in the direction of the current and curl your fingers around—your fingers point in the same direction as the magnetic field created by the current.
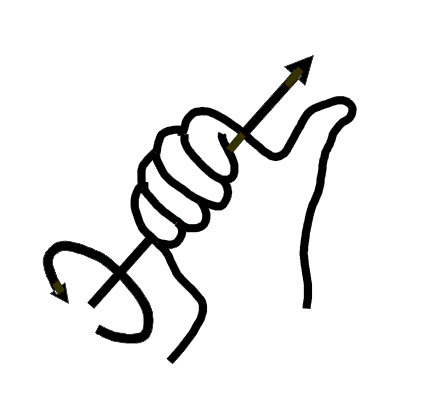
Thumbs up, dude.
(Source)
Ampère's Law
We can find the strength of the magnetic field B created by a current I using Ampère's Law. This is very similar to Gauss' Law for the electric field created by a charge—for Gauss' Law, we drew a Gaussian surface enclosing our charge and looked at the electric field lines hitting the surface. In Ampère's Law, we draw an Ampèrian loop around our current-carrying object.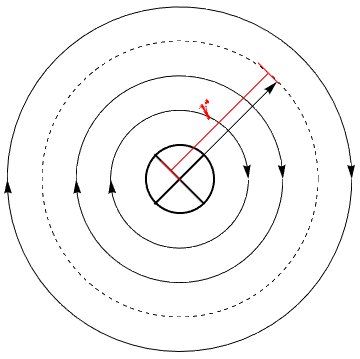
The magnetic field created by the enclosed current is:
Bl = μ 0I
Here, l is the length of the section of our loop that is parallel to the magnetic field. The symbol μ0 represents the magnetic permeability of free space. It is exactly analogous to electric permittivity—μ0 represents the ease a magnetic field has moving through vacuum (or, as with ε0, air). Permeability is measured in N/A2, where:
μ0 = 4π × 10-7 N/A2
This means that magnetic fields are measured in

For the case of an Ampèrian loop drawn around a current carrying wire, both the loop and the field are circular, so the loop is parallel to the field at every single point. In this case, l is equal to the entire circumference of the loop and we can write:
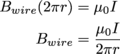
Electromagnetic Radiation
The similarities between magnetic permeability and electric permittivity are no coincidence. Any charged particle can emit electromagnetic radiation, a form of electric and magnetic energy that travels like a wave through space.Electromagnetic radiation comes in many different forms—in fact, many different forms you've heard of: radio waves, microwaves, infrared light, visible light, ultraviolet light, X-rays, and gamma rays. These are all forms of electromagnetic energy. And in vacuum, each of them travels at the speed of light (c). Well, guess what the speed of light is equal to:

In future chapters, we'll learn more about what a wave is in physics and how waves move. For now, let's just say waves are what happen when a charged particle listens to too many Beach Boys albums. The important things to note are:
• Electromagnetic waves are a form of electromagnetic energy that is radiated from charged particles.
• The amount of electromagnetic energy in a wave is given by its frequency, a measure of how quickly the wave moves up and down. Frequency is measured in inverse seconds, or hertz (Hz).
We group waves by frequency in a chart called the electromagnetic spectrum.
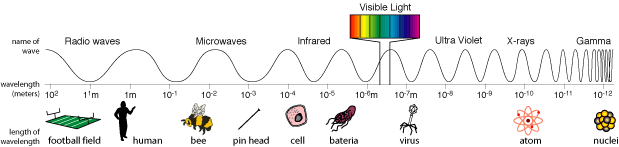
Everything you'd like to see…and then some.
(Source)
The higher the frequency, the more energy is in the wave. Radio waves are harmless, but microwaves can cook food, X-rays can see through skin and muscle, and gamma rays—well, Hulk smash. Sitting in the middle of the EM spectrum is visible light, how humans perceive the world around us. Low frequency light looks red or orange, while higher frequency light looks blue or violet.